Effects of salinity on the growth, physiological and biochemical components of microalga Euchlorocystis marina
- College of Fisheries, Guangdong Ocean University, Zhanjiang, China
Euchlorocystis marina, a new marine species of the genus Euchlorocystis discovered in 2022, has the potential to improve the water quality in mariculture ponds. However, the effects of salinity on the growth, physiology, and biochemical composition of these algae are not well understood. In this study, changes in physiological and biochemical indices such as cell density, photosynthetic pigment, polysaccharide, and lipid content of E. marina under different salinity treatments were analyzed. The results showed that the highest cell density was observed at a salinity of 15‰. The lowest photosynthetic pigment content was observed at a salinity of 60‰, and the highest polysaccharide and lipid content was observed at a salinity of 60‰. These results indicated that lower salinity was more conducive to E. marina reproduction and growth. E. marina can accumulate polysaccharides and lipids in high salinity environments. This study provides new information for understanding the salinity adaptation strategies of E. marina and has practical significance for its development and utilization.
1 Introduction
Over the past few decades, population and economic growth have led to increasingly serious problems, such as eutrophication and water pollution in coastal water bodies, hindering the sustainable development of the mariculture industry (Kang et al., 2021). Microalgae, which are the natural producers of organic matter and inorganic matter in the aquatic environment, play an important role in stabilizing micro-ecosystems and maintaining healthy water quality in aquaculture ponds (Salam et al., 2016; Esteves et al., 2022). They can absorb carbon dioxide through photosynthesis to produce oxygen and increase the dissolved oxygen levels in the water, as well as purify water by absorbing and transforming excess nutrients (Cheng et al., 2023). To address the negative impacts of unregulated human activities on aquaculture environments, there is growing interest in technology that uses direct artificial culture of green algae to improve the aquatic environment in aquaculture facilities in China.
In Southern China, there are many mariculture ponds that cover an estimated 165,000 km (Liu et al., 2023). Huang (2013) observed that salinity levels in these aquaculture ponds fluctuate significantly over time. A decrease in salinity can lead to an increase in the dominance of toxic microalgal species such as cyanobacteria, which can destabilize the aquaculture ecosystem and result in water quality deterioration and disease outbreaks (Xie et al., 2003; Yang et al., 2019; Lian et al., 2024). Additionally, during droughts, the salinity levels of some ponds may increase to over 40‰. These drastic fluctuations in salinity can affect the structure of the phytoplankton community in ponds, which can disrupt the ecological balance. Studies have shown that the presence of green algae with wide salinity tolerance can effectively inhibit the growth of pond cyanobacteria and improve the stability of aquaculture ecosystems (Wang et al., 2020). Therefore, constructing a mariculture pond environment with salt-tolerant microalgae as the dominant population can effectively reduce the risk of ecological imbalances due to salinity fluctuations.
Oocystaceae is a widely distributed green alga that possesses complex biological characteristics and is capable of adapting to various environmental conditions due to its long-term evolution (Li et al., 2022). Some Oocystaceae species have the potential to improve aquatic environments (Liu et al., 2018a, 2020). and wastewater treatment (Ajala and Alexander, 2020) because of their capacity to absorb nitrogen, P, and CO2 (Ajala and Alexander, 2020; Chuka et al., 2020). Furthermore, they can adapt to various environmental conditions (Takeuchi et al., 1992; Huang et al., 2002). At present, the majority of reported Oocystaceae species are freshwater species, with only a few marine species being taxonomically accepted, such as Oocystis submarina, Oocystis marina, and Euchlorocystis subsalina (Liu et al., 2018b). Euchlorocystis marina is a newly isolated marine green algal species belonging to the Oocystaceae family (Li et al., 2022). Their biological characteristics and ecological functions are similar to those of Oocystis and have the potential to improve the water quality of aquaculture ponds. However, the effects of salinity on the growth, physiology, and biochemical composition of algae are poorly understood. The salinity level in the mariculture ponds in southern China typically falls between 25–35 ‰. Nevertheless, under conditions of drought, this level can exceed 40 ‰ and may decrease below 20 ‰ during periods of rainfall. Therefore, this study aimed to analyze the changes in growth and physiological and biochemical indices under different salinity conditions to understand the salinity adaptability of E. marina. The results of this study provide valuable information for the development and utilization of this alga.
2 Materials and methods
2.1 Microalgal strain
E. marina (Figure 1) was isolated from a mariculture shrimp pond in Zhanjiang and was inoculated and provided by the Laboratory of Algae Resources Development and Aquaculture Environmental Ecological Restoration of the College of Fisheries, Guangdong Ocean University (accession number OM413748; NCBI).
2.2 Culture conditions
The strains were maintained at 15 μmol photons m−2 s−1 in Zhanshui 107–13 seawater medium. E. marina working cultures were cultured and reproduced in f/2 culture medium with artificial seawater under continuous light (30 μmol photons m−2 s−1), aeration (0.5 L min−1), and temperature (25 ± 1°C) in a 1 L Erlenmeyer flask. The aeration was stopped a day before the experiment to allow the algal suspension to settle naturally, and the supernatant was removed to collect the concentrated algal solution. The algal solution was centrifuged (at 25°C and 4500 r min-1 for 10 min), the supernatant was removed, and the algal cells were washed and resuspended in 1 L of artificial seawater at an initial pH of 8 and 25‰ salinity. The pH and salinity of the culture were adjusted using a YSI model 63 multi-parameter meter (Yellow Springs Instrument Co., USA).
Algal sludge was then added to the f/2 culture medium at salinity levels of 15, 30, 45, and 60 ‰ to inoculate an initial OD680 value of 0.5. The modified f/2 medium was prepared with the following composition: NaNO3 (75 mg), KH2PO4 (5 mg), Na2SiO3–9H2O (20 mg), and F/2 trace element solution (1 mL) per liter of double-distilled water. The f/2 trace element solution comprised C10H14N2Na2O8 (4160 mg), FeCl3·6H2O (3150 mg), MnCl2·4H2O (180 mg), ZnSO4·4H2O (22 mg), CuSO4·5H2O (10 mg), H4MoNa2O6 (6 mg), and CoCl2·6H2O (4160 mg) per liter of double-distilled water. Different levels of salinity were achieved by adding appropriate amounts of distilled water or NaCl to the seawater and then adjusting the concentrations to the desired level. The experiment was carried out in triplicate for each group and cultured under growth conditions of temperature (25 ± 2°C), light intensity (30 ± 2 μmol photons m-2 s-1), and photoperiod (12:12 h, light: dark).
2.3 Algal cell density
5 mL of algal suspension was taken every two days and measured at an optical density of 680 nm (OD680) using a UV2450 spectrophotometer. The algal cell density was calculated based on the annotation curve of the OD680 value (Xie et al., 2020).
The standard curve is:
Correlation coefficient R2 = 0.9968, where Y is the value of OD680 and X is the cell density (×104 cells mL-1).
2.4 Photosynthetic pigment content determination
The photosynthetic pigments in E. marina were extracted and determined according to the method described by Ren (2021). Briefly, 5 mL of algae solution of various salinity groups was taken after shaking the solution thoroughly and centrifuged at 5,000 r min-1 for 15 min. The supernatant culture solution and an equal amount of 1×PBS (pH 7.2) using phosphate buffer salt solution were added, mixed thoroughly, and centrifuged at 5,000 r min-1 for 15 min, after which the supernatant was removed. The centrifuge tubes were wrapped in tin foil and placed in a refrigerator at -20°C for 12 h in the dark. 5 mL of 95% ethanol was preheated at 80°C for 30 min was added quickly and thoroughly mixed, and placed in an 80°C water bath for 5 min. The solution was then wrapped in tin foil and placed in the dark at room temperature for 4 h. Subsequently, the solution was centrifuged at 5000 r min-1 for 15 min, and the optical density of the supernatant was measured at 470, 649, and 665 nm using a UV2450 UV spectrophotometer. Pigment content was calculated using the equation described by Lichtenthaler and Wellburn (1983).
Where Chl a is chlorophyll a, Chl b is Chlorophyll b, and OD is measured absorbance values at different wavelength.
2.5 Determination of chlorophyll fluorescence parameters
The maximum photochemical efficiency of PSII (Fv/Fm) and the actual photochemical efficiency of PSII (ΦPSII) were measured using an FMS-2 pulse-modulated fluorometer (Hansatech, UK). The steady-state fluorescence (Fs) and light-adapted maximum fluorescence (Fm) values were recorded when the chlorophyll fluorescence reached a steady-state level in the light, and ΦPSII = 1 − (Fs/Fm) · (Fv/Fm) was measured after 30 min of dark adaptation (Ren et al., 2020).
2.6 Polysaccharide content determination
A standard curve was prepared according to the phenol-sulfuric acid method (Han, 2018). Briefly, 100 μg mL-1 glucose was diluted to different concentrations (0, 40, 80, 120, 160, and 200 μL). Distilled water was added to the diluted solution to 200 μL, followed by the addition of 200 μL of 5% phenol, and subsequent mixing. 1 mL of concentrated H2SO4 was added, mixed, and left for 30 min. A total of 250 μL was added to a 96-well cell culture plate and the absorbance of the solution was measured at 490 nm using a Synergy 2 microplate reader. The standard curve of glucose was
The correlation coefficient (R2) was 0.9994, where Y is the absorbance and X is the glucose concentration.
The polysaccharide content of microalgae was determined according to the method described by Wang et al. (2012). The algal solution (3 mL) was centrifuged at 5000 r min-1 for 15 min, the supernatant was removed, and an equal amount of 1×PBS phosphate buffer salt solution was added and thoroughly mixed. The supernatant was removed after centrifugation at 5000 r min-1 for 15 min, 1 mL of 0.305 mol L-1 NaOH solution was added to wash the algae sludge, and the sample was then incubated in a water bath at 58°C for 68 min. After centrifugation at 5000 r min-1 for 15 min, 200 μL of supernatant was transferred to a 2 mL centrifuge tube, 200 μL of 5% phenol reagent and 1 mL of concentrated H2SO4 were added and thoroughly mixed, and the mixture was allowed to settle for 30 min. A total of 250 μL was added to a 96-well cell culture plate and the absorbance of the solution was measured at 490 nm using a Synergy 2 microplate reader. Polysaccharide content was calculated using the glucose standard curve equation.
2.7 Lipid content determination
Using the method described by Wang et al. (2012), an appropriate amount of algal solution was centrifuged (5000 rpm for 10 min) and suspended in 1× PBS to an OD680 value of 0.8. Subsequently, 1 mL of the solution was transferred to a 2 mL centrifuge tube and centrifuged at 5000 rpm for 10 min. The supernatant was removed and the algal cell pellet was washed twice with 1× PBS. Then, 1 mL of 20% dimethyl sulfoxide solution was added, and the mixture was incubated in a water bath at 38°C for 18 min. After adding 20 μL of Nile Red dye (0.1 mg/mL acetone solution) and mixed, the sample was stained for 5 min. 250 μL of the sample was placed in a 96-well microplate and its absorbance value was determined using a multi-mode microplate reader. The wavelength was set at 480 nm, and the fluorescence intensity at 570 nm was measured.
2.8 Statistical analysis
One-way ANOVA with Duncan’s test (post hoc) was used to conduct statistical analysis with the aid of the SPSS for Windows statistical software package (IBM SPSS v26.0; Chicago, USA). The significance level was set at P< 0.05, and the results are expressed as mean ± SD.
3 Results
3.1 Effect of salinity on the cell density of E. marina
The effects of the different salinity treatments on E. marina cell density are shown in Figure 2. Salinity significantly affected E. marina cell density (P< 0.05). E. marina grew normally at salinity of 15‰, 30‰, 45‰, and 60‰. 15‰ salinity showed a maximum cell density of 507.96×10–4 cells mL-1 compared with 60‰ salinity which showed a minimum cell density (383.84×10–4 cells mL-1). The cell density of E. marina at 15 ‰ and 30 ‰ salinity was higher. However, the cell density of E. marina was significantly (P< 0.05) higher at a salinity of 15‰. The cell density at all salinity levels increased from the second day to the final day of cultivation.
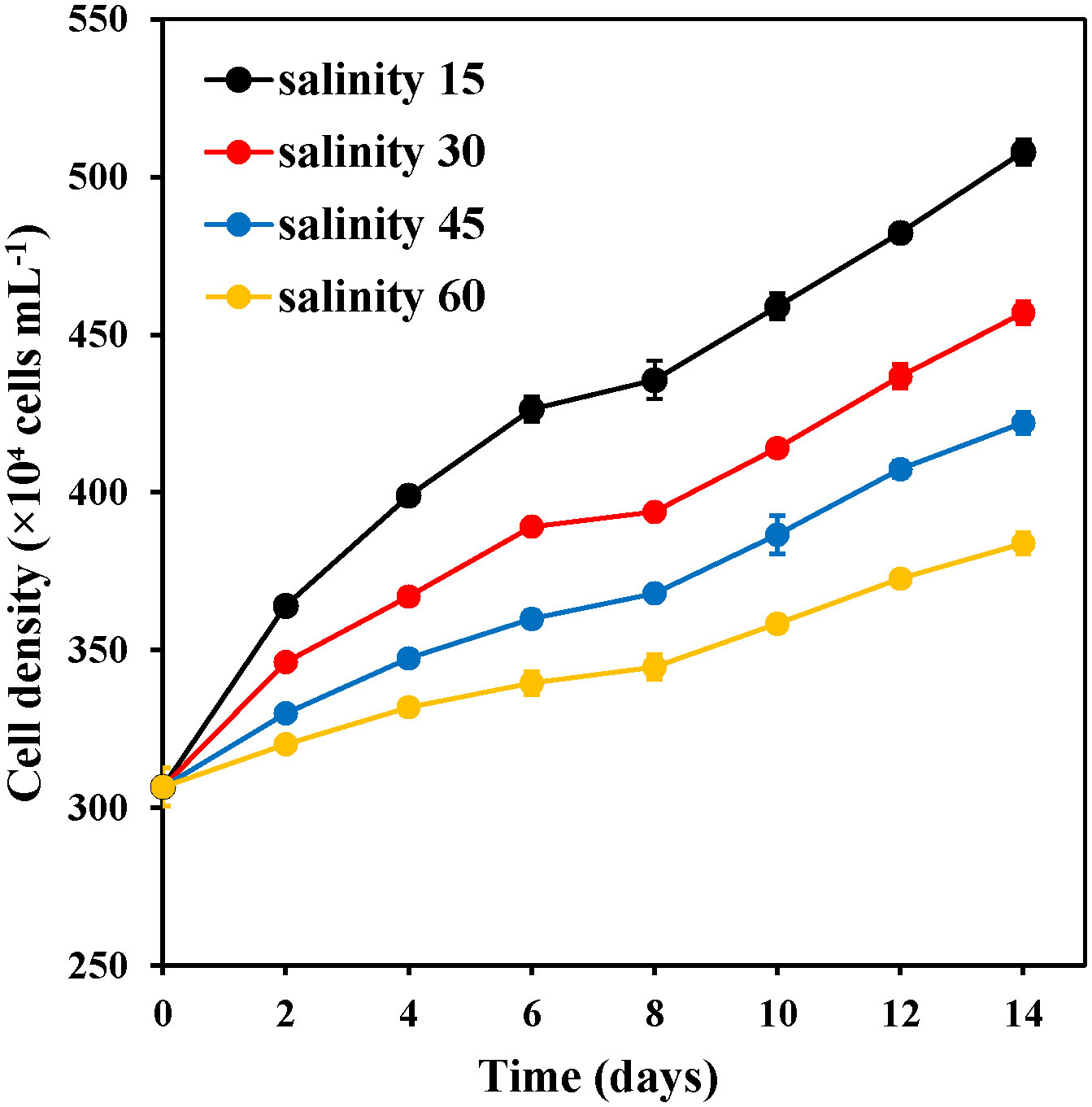
Figure 2 Changes in the cell density of E. marina at different levels of salinity (mean ± SD, n = 3).
3.2 Effect of salinity on the photosynthetic pigment content of E. marina
3.2.1 The total chlorophyll content and chlorophyll content
Figure 3 shows the pigment contents of E. marina at different salinity levels. The salinity of 60‰ caused a significant decline in the pigment content of E. marina during day 0–4 for chlorophyll a (Figure 3A), day 0–2 for chlorophyll b (Figure 3B) and day 2–4 for carotenoid (Figure 3C), which resulted in the lowest pigment content among the groups. 30‰ salinity showed significantly (P< 0.05) higher chlorophyll a and b and carotenoid content with 1.7995 pg cell-1, 0.4824 pg cell-1, and 0.4294 pg cell-1, respectively, compared with 45 ‰ and 60 ‰ salinity. However, there was no significant (P > 0.05) evidence between salinity of 30‰ and 15‰.
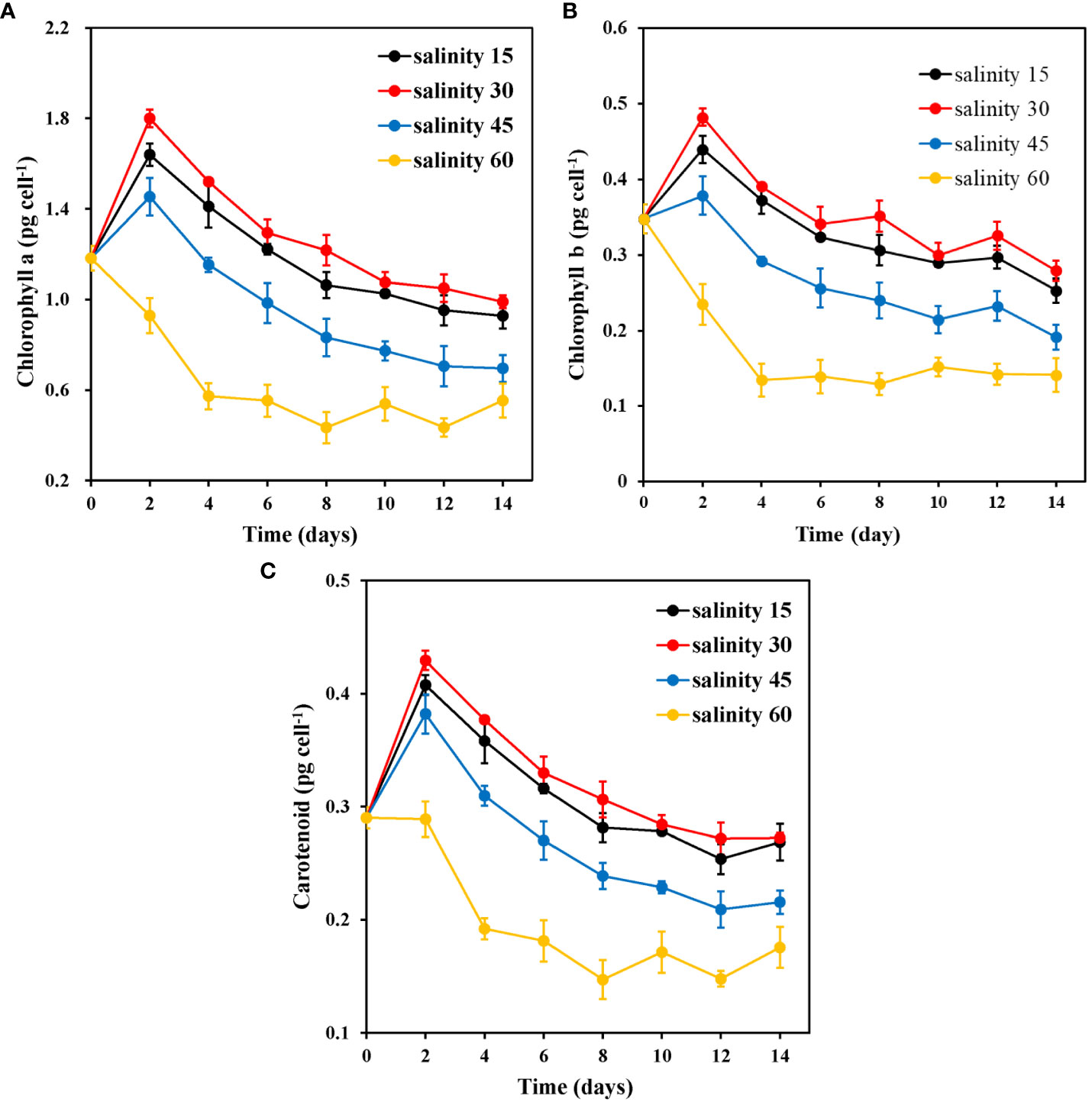
Figure 3 Changes in chlorophyll a (A), chlorophyll b (B), and carotenoid (C) content of E. marina at different levels of salinity (mean ± SD, n = 3).
3.2.2 Photosynthetic efficiency (ФPSII and Fv/Fm)
The ФPSII and Fv/Fm values of E. marina were affected by salinity levels (Figure 4). There were significant differences in ФPSII at various salinity levels on days 4 and 14. The ФPSII efficiency decreased by 12.0%, 14.5%, 16.6%, and 14.0% under 15, 30, 45, and 60‰ salinity, respectively, on day 8 (Figure 4A). However, on the final day of cultivation, ФPSII efficiency increased significantly by 6.1%, 3.5%, 1.2%, and 12.7% under 15, 30, 45, and 60‰ salinity respectively (Figure 4A). The changes in Fv/Fm, an indicator of ФPSII activity, were consistent with those in ФPSII in the various salinity groups on day 8 (Figure 4B). For example, the efficiency of Fv/Fm decreased by 10.3%, 9.5%, 10%, and 9.6% under 15, 30, 45, and 60‰ salinity, respectively, and showed a trend similar to that of ФPSII (Figure 4A).
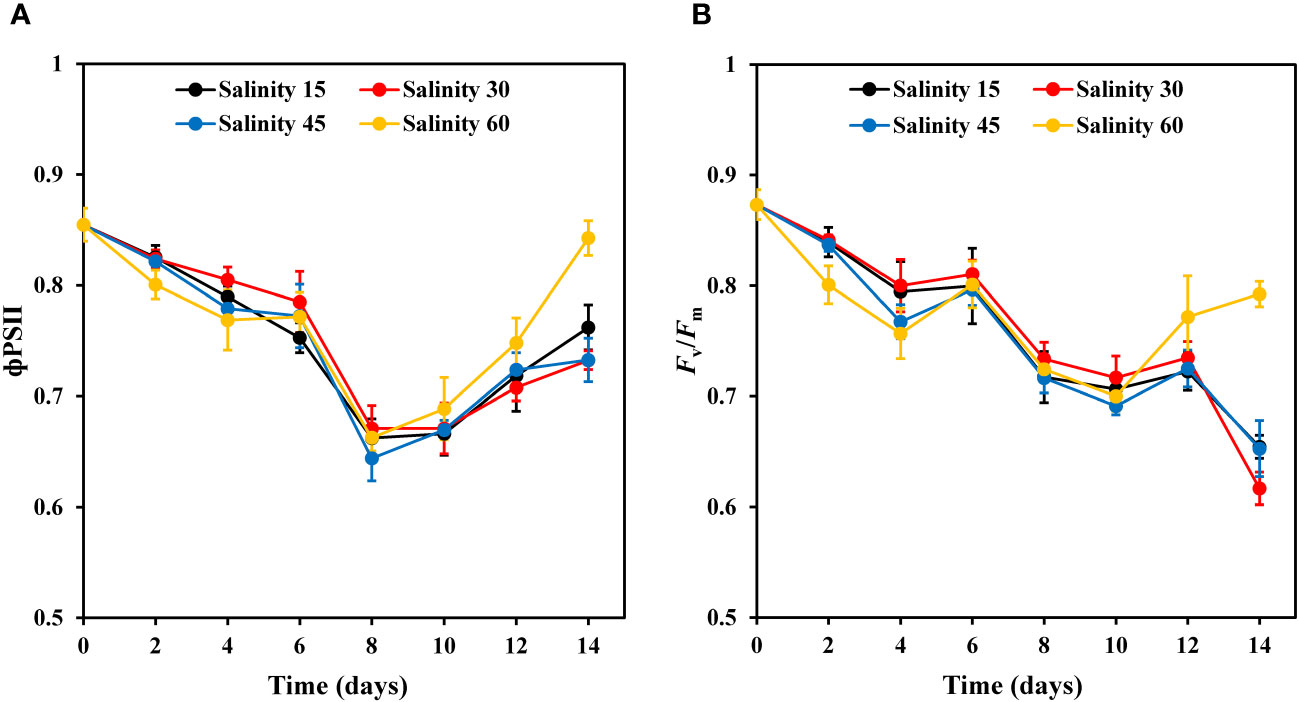
Figure 4 Changes in ФPSII (A) and Fv/Fm (B) of E. marina at different levels of salinity (mean ± SD, n = 3).
3.3 Effect of salinity on the polysaccharide content of E. marina
The polysaccharide content of E. marina significantly increased with increasing salinity (Figure 5). Salinity levels of 15‰ and 30‰ exhibited a swift decrease from days 0 to 2, while the salinity 45‰ group maintained its initial level after day 2. The polysaccharide content in the 60‰ group was significantly (P< 0.05) higher (745.63 pg cell-1) on the 8th day of cultivation than the low polysaccharide content observed in the 15‰ group during the same period.
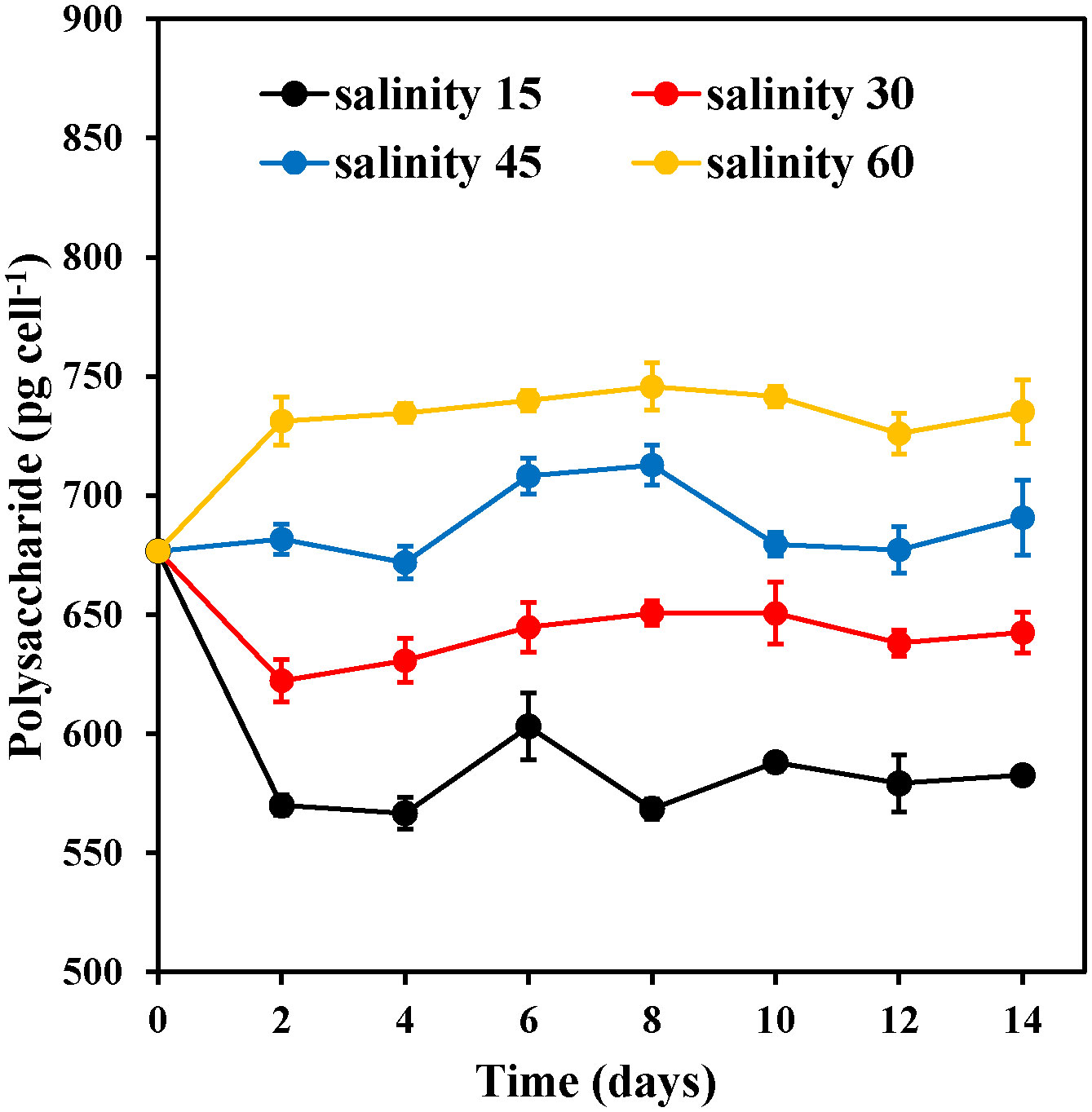
Figure 5 Changes in the polysaccharide content of E. marina at different levels of salinity (mean ± SD, n = 3).
3.4 Effect of salinity on the lipid content of E. marina
Fluorescence intensity was used to measure the variations in the lipid content of E. marina among the different salinity groups. The results showed that salinity significantly affected the lipid content of the E. marina. The lipid content of all salinity groups fluctuated from day 2 to day 8, as shown in Figure 6; however, the lipid content of E. marina continued to increase after the 10th day. The lipid content increased with increasing salinity levels, with the maximum lipid content of E. marina observed at 60‰ salinity, which was significantly higher (P< 0.05) than that of the other salinity groups. Additionally, the 15‰ and 30‰ salinity groups had lower lipid content than the 45‰ and 60‰ salinity groups.
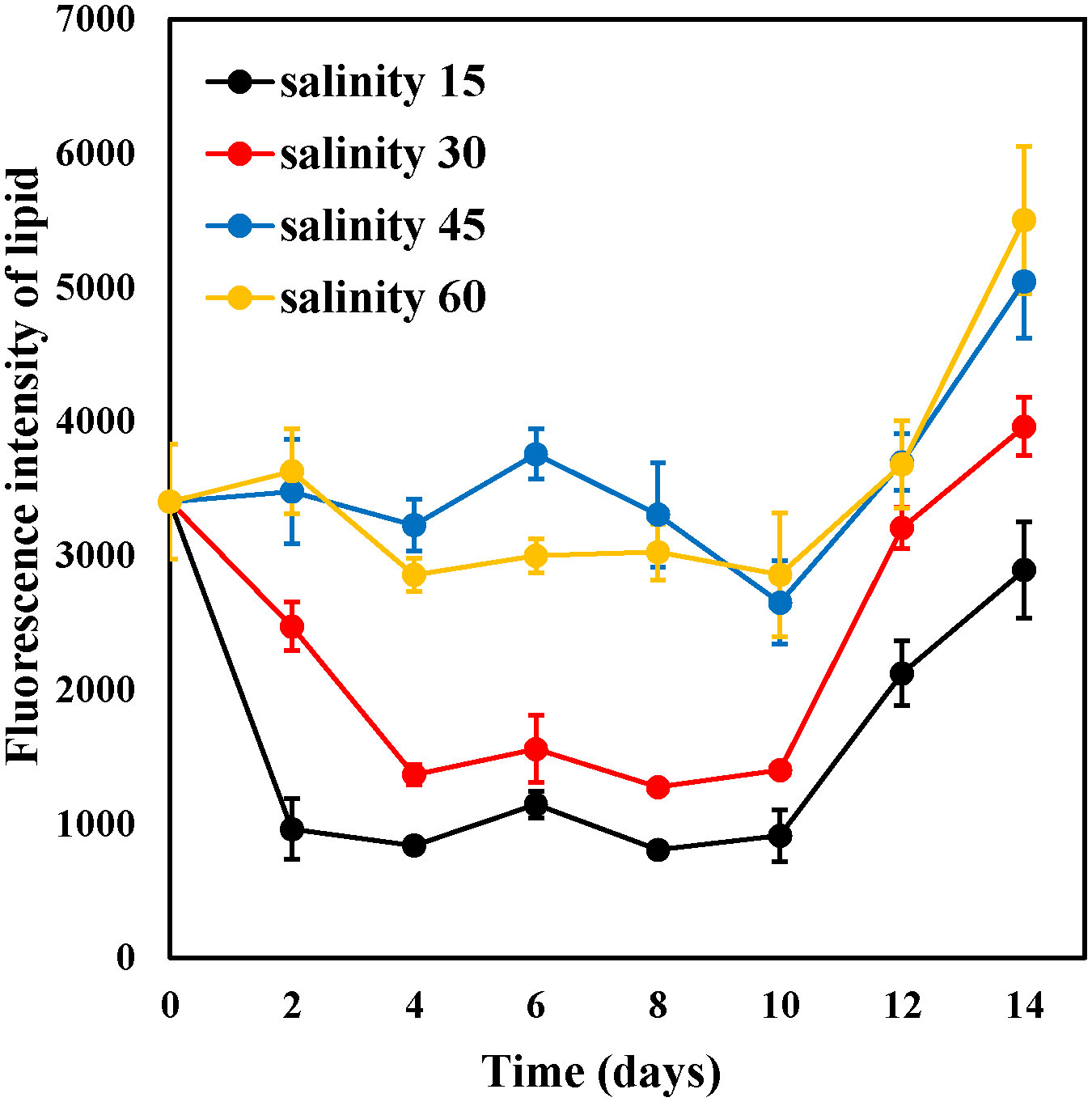
Figure 6 Changes in the fluorescence intensity of lipid content of E. marina at different levels of salinity (mean ± SD, n = 3).
4 Discussion
Salinity plays a crucial role in shaping the structure and function of aquatic ecosystems (Smyth and Elliott, 2016). The effects of changing salinity levels in aquaculture ponds are primarily determined by the physiology and tolerance of organisms, and their ability to cope with fluctuations in salinity. Marine microalgae have a specific range of adaptability to different salinity levels. In this study, E. marina was divided and multiplied at a salinity range of 15– 60 ‰. Maximum growth of E. marina was obtained under 15 ‰. However, 15–30 ‰ can serve as the optimum salinity range for growth and biomass production. This demonstrates that algal cell growth and metabolic activity were supported at the salinity of 15–30 ‰ (Rasool et al., 2013). A similar salinity range (25–35 ppt) was reported for the maximum growth of T. suecica by Talukdar et al. (2012) and 1.5–2.5% for Isochrysis galbana by Laing and Utting (1981). Excessive levels of salinity may result in a rapid decrease in the cellular water content, which increases the concentration of salts within the cells. This can impede cellular metabolism, nutrient uptake, and utilization which negatively affects the growth of E. marina. However, E. marina was found to be capable of maintaining some degree of growth even at high salinities of 60‰, suggesting that it is a salt-tolerant species.
As previously reported by Zhang et al. (2010), the salinity also affects the pigment content of microalgae. High salinity can hinder photosynthetic activity in microalgae and Yao et al. (2013) found that, high salinity affected the photosynthetic activity in Tetraselmis subcordiformis. The findings of this study suggest that, except for the salinity 60‰ group, the other salinity groups accumulated photosynthetic pigments during the initial two days. This indicates that E. marina experienced stress at salinity of 60‰. The result agrees with what was reported by Sedjati et al. (2019). They recorded higher chlorophyll a and b content of Dunaliella salina at low level of salinity (20 ‰). Additionally, Park et al. (2015) discovered that the overall chlorophyll content of Dunaliella tertiolecta decreased significantly under high salinity conditions. Pancha et al. (2015) also observed a substantial reduction in chlorophyll a, chlorophyll b, and total carotenoid contents in Scenedesmus sp. when the NaCl concentration in the medium was increased. Similar to previous findings, the results of the present study showed a significant decrease in chlorophyll a, b, and total carotenoid content of E. marina at higher salinity ranges. Limitations of photosynthetic electron transport in high-salt environments may impede the synthesis of photosynthetic pigments in algal cells, resulting in reduced chlorophyll production (Zhang et al., 2010; Ren et al., 2020). From day 2, the photosynthetic pigment content of each salinity group gradually decreased, and the number of cells decreased with an increase in cultivation time. This could be a result of the limited amount of light energy channeled into the photosystem pathway, which in turn decreases the accumulation of photosynthetic pigment synthesis (Srivastava and Goud, 2017). PSII is considered to be one of the most important components of the electron transport chain. This component is measured by its ability to utilize absorbed light for photochemical processes. The Fv/Fm ratio, which is the ratio of variable to maximum fluorescence, is a useful indicator of the efficiency of photochemistry in PSII, and can reveal the level of stress experienced by plants. In the present study, we found that ФPSII and Fv/Fm values decreased with an increase in salinity concentration (45 and 60‰) during the first four days of culture, indicating that salinity stress resulted in a decrease in PSII activity. Our findings align with those of Liang et al. (2014), indicating that the Fv/Fm values for P. tricornutum are higher at salinities ranging from 20 to 40 psu. Additionally, for C. gracilis, the Fv/Fm values were higher at salinities of 5–40 psu compared to those at 1 psu or 50–70 psu.
Different salinity levels can affect the accumulation and consumption of microalgal carbohydrates. When algal cells are exposed to salinity, certain regulatory processes are activated, such as the regulation of the uptake and export of ions through the cell membrane (Allakhverdiev et al., 2000), restoration of expansion pressure, and accumulation of osmoprotective solutes and stress proteins (Talebi et al., 2013), which maintain the cells in a stable growth state. Zou (2018) observed that the polysaccharide content of Spirulina platenis was significantly elevated and maintained at a high level under saline conditions, suggesting that polysaccharides might be involved in osmoregulation. Polysaccharide not only plays a positive role in the regulation of cellular osmotic pressure, but also participates in the formation of biofilm (Rinaudi and González, 2009). Qurashi and Sabri (2012) concluded that increased secretion of total cellular sugars under high salt stress promotes biofilm formation and protects cells. According to Tewari and Arora (2014), higher concentrations of NaCl affect the composition of extracellular polymers and increase rhamnose and trehalose levels, which can help microorganisms to tolerate salt stress. In this study, the polysaccharide content of E. marina increased with increasing salinity. This showed that, to adapt to the environment of increased salinity, algal cells synthesize polysaccharides or maintain high levels of polysaccharide content to maintain osmotic balance inside and outside cells. Increased extracellular polysaccharides maintain optimal levels of intracellular sodium content and antioxidant enzyme activities (Liu et al., 2017), which may help E. marina adapt to high-salinity environments. Overall, maintaining a high polysaccharide content in high-salinity environments has a positive effect on the osmoregulatory capacity of E. marina and the formation of biofilms with protective functions.
The lipid content of microalgae can be influenced by various environmental factors such as stress. Research has shown that increasing salinity levels can lead to an increase in both lipid and fatty acid contents in microalgae (Feng and Guo, 2009). In this study, we observed that E. marina exposed to salinity levels of 45‰ and 60‰ had higher lipid content than those exposed to 15‰ and 30‰. This finding is consistent with a previous study that reported the highest lipid content of I. galbana at a salinity level of 40 ppt (15.68 ± 0.58% of dry weight) (Haris et al., 2022). It is also known that salt stress conditions cause the degradation of starch and the accumulation of lipids in microalgae cells (Zhang et al., 2018). Microalgae accumulate intracellular lipids as long-term energy sources in response to salt stress (Ines et al., 2016). This accumulation, particularly of neutral lipids, helps to maintain membrane integrity under salt stress, reducing cell membrane osmotic pressure and fluidity (Ji et al., 2018). Srivastava and Goud (2017) reported that under salt stress, algae preferred the synthesis of neutral lipids, and that elevated neutral lipid content improved the ability of algal cells to cope with unfavorable environments. Kan et al. (2012) showed that the increase in the proportion of saturated fatty acids in organisms under salt stress can effectively reduce the fluidity of biological membranes, which in turn reduces the damage of cell membranes. Lipid accumulation in algal cells is a survival strategy in response to salt stress, enabling algal cells to respond to increases in osmotic pressure and to maintain cell membrane fluidity and integrity.
5 Conclusion
In aquaculture activities, the salinity of mariculture ponds fluctuates significantly. Frequent fluctuations in salinity have a significant impact on the community structure of planktonic microalgae in the culture system. Therefore, the adaptability of microalgae to salinity is an important parameter affecting the success of the construction of ideal plankton communities in mariculture ponds. This study investigated the effects of salinity on the growth, physiology, and biochemical composition of E. marina. Our findings showed that cells multiplied at a faster rate at a salinity of 15. Algal cells can synthesize photosynthetic pigments more efficiently at a salinity of 30, resulting in faster growth. At a salinity of 60, the cells could cope with the high-salinity environment by accumulating and preserving polysaccharides and lipids. E. marina microalga exhibits a broad range of salinity tolerance, making it a promising candidate for regulating the environment of mariculture ponds. However, there is a need for further investigation of the impact of fluctuating salinity levels on the uptake and metabolism of excess nutrients in the water of aquaculture ponds by this microalga.
Data availability statement
The original contributions presented in the study are included in the article/supplementary material. Further inquiries can be directed to the corresponding author.
Author contributions
YP: Data curation, Formal Analysis, Investigation, Writing – original draft. DA: Writing – original draft, Writing – review & editing. MD: Data curation, Formal Analysis, Investigation, Methodology, Writing – original draft. NZ: Funding acquisition, Methodology, Writing – original draft. XH: Resources, Writing – original draft. CL: Resources, Writing – original draft. FL: Formal Analysis, Funding acquisition, Methodology, Project administration, Resources, Writing – original draft, Writing – review & editing.
Funding
The author(s) declare that financial support was received for the research, authorship, and/or publication of this article. This research was mainly supported by the Program for Scientific Research Start-up Funds of Guangdong Ocean University (grant number 060302022103) and Natural Science Foundation of China (grant number 32102796).
Conflict of interest
The authors declare that the research was conducted in the absence of any commercial or financial relationships that could be construed as a potential conflict of interest.
Publisher’s note
All claims expressed in this article are solely those of the authors and do not necessarily represent those of their affiliated organizations, or those of the publisher, the editors and the reviewers. Any product that may be evaluated in this article, or claim that may be made by its manufacturer, is not guaranteed or endorsed by the publisher.
References
Ajala S. O., Alexander M. L. (2020). Assessment of chlorella vulgaris, scenedesmus obliquus, and oocystis minuta for removal of sulfate, nitrate, and phosphate in wastewater. Int. J. Energy Environ. Engineering. 11, 311–326. doi: 10.1007/s40095-019-00333-0
Allakhverdiev S. I., Sakamoto A., Nishiyama Y., Inaba M., Murata N. (2000). Ionic and osmotic effects of naCl-induced inactivation of photosystems I and II in synechococcus sp. Plant Physiol. 123, 1047–1056. doi: 10.1104/pp.123.3.1047
Cheng P., Shan S., Zhu Z., Liu K., Namsaraev Z., Dubovskiy I., et al. (2023). The role of microalgae culture modes in aquaculture: A brief opinion. Front. Bioeng. Biotechnol. 11. doi: 10.3389/fbioe.2023.1196948
Chuka O. D., Ogbonna J., Borowitzka M. A., Michael A. (2020). Screening, acclimation and ammonia tolerance of microalgae grown in food waste digestate. J. Appl. Phycology. 32, 3775–3785. doi: 10.1007/s10811-020-02276-0
Esteves A. F. F., Soares S. M. M., Salgado E. M. M., Boaventura R. A. R., Pires J. C. M. (2022). Microalgal growth in aquaculture effluent: coupling biomass valorisation with nutrients removal. Appl. Sci-Basel. 12, 12608. doi: 10.3390/app122412608
Feng L., Guo Y. (2009). Effects of salinity on the total lipids contents and fatty acids composition of 4 strains of marine green algae. J. Tianjing Univ. Sci. Technology. 24, 22–28. doi: 10.3969/j.issn.1672–6510.2009.04.006
Han Q. (2018). Effects of ecological factors on polysaccharide extraction and metabolism of oocystis borgei. [Master dissertation]. Guangdong Ocean University, zhanjiang.
Haris N., Manan H., Jusoh M., Khatoon H., Katayama T., Kasan N. A. (2022). Effect of different salinity on the growth performance and proximate composition of isolated indigenous microalgae species. Aquaculture Rep. 22, 1–15. doi: 10.1016/j.aqrep.2021.100925
Huang X. H. (2013). Culture Pollution and Ecological Control Mechanisms using Planktonic microalgae in high level Prawn Ponds. [Doctoral Dissertation]. Donghua University, Shanghai, shanghai.
Huang X. H., Li C. L., Liu C. W., Zeng D. S. (2002). Ecological conditions for the culture of oocystis borgei. J. Guangdong Ocean University. 22, 8–12.
Ines B., Haifa C., Fatma R., Sami S., Abdelhafidh D. (2016). Salinity stress increases lipid, secondary metabolites and enzyme activity in Amphora subtropica and Dunaliella sp. for biodiesel production. Bioresource Technology. 218, 816–825. doi: 10.1016/j.biortech.2016.07.022
Ji C., Xue M., Hao J., Wang X., Xue J., Cui H., et al. (2018). Analysis of bZIP Transcription Factor Family and Their Expressions under Salt Stress in Chlamydomonas Reinhardtii. Int. J. Mol. Sci. 19, 2800. doi: 10.3390/ijms19092800
Kan G. F., Shi C. J., Wang X. F., Xie Q. J., Wang M., Wang X. L., et al. (2012). Acclimatory responses to high-salt stress in chlamydomonas (Chlorophyta, chlorophyceae) from Antarctica. J. Oceanography. 31, 116–124. doi: 10.1007/s13131-012-0183-2
Kang B., Pecl G. T., Lin L., Lin L., Sun P., Zhang P., et al. (2021). Climate change impacts on China’s marine ecosystems. Rev. Fish Biol. Fisheries 31, 599–629. doi: 10.1007/s11160-021-09668-6
Laing I., Utting S. D. (1981). The influence of salinity on the production of two commercially important unicellular marine algae. Aquaculture. 21, 79–86. doi: 10.1016/0044-8486(80)90127-1
Li F., Dong M., Zhang N., Zhang Y. L., Li Q., Qian Z., et al. (2022). Euchlorocystis marina sp. nov. (Oocystaceae, Trebouxiophyceae), a new species of green algae from a seawater shrimp culture pond. Diversity. 14, 119. doi: 10.3390/d14020119
Lian C., Xiang J., Cai H., Ke J., Ni H., Zhu J., et al. (2024). Microalgae inoculation significantly shapes the structure, alters the assembly process, and enhances the stability of bacterial communities in shrimp-rearing water. Biology 13, 54. doi: 10.3390/biology13010054
Liang Y., Sun M., Tian C., Cao C., Li Z. (2014). Effects of salinity stress on the growth and chlorophyll fluorescence of Phaeodactylum tricornutum and Chaetoceros gracilis (Bacillariophyceae). Biotanica Marina. 57, 469–476. doi: 10.1515/bot-2014-0037
Lichtenthaler H. K., Wellburn A. R. (1983). Determinations of total carotenoids and chlorophylls a and B of leaf extracts in different solvents. Biochem. Soc. Trans. 11, 591–592. doi: 10.1042/bst0110591
Liu X., Cui L., Li S., Jiang K., Yuan X., Yu X., et al. (2023). China fishery statistical yearbook. Beijing: China Agriculture Press.
Liu M., Huang X. H., Li C. L., Gu B. (2020). Study on the uptake of dissolved nitrogen by Oocystis borgei in prawn (Litopenaeus vannamei) aquaculture ponds and establishment of uptake model. Aquacult Int. 28, 1445–1458. doi: 10.1007/s10499-020-00534-z
Liu M., Huang X. H., Zhang R., Li C. L., Gu B. H. (2018a). Uptake of urea nitrogen by oocystis borgei in prawn (Litopenaeus vannamei) aquaculture ponds. Bull. Environ. Contamination Toxicology. 101, 586–591. doi: 10.1007/s00128-018-2450-1
Liu X. D., Luo Y. T., Li Z. F., Wang J. M., Wei J. H. (2017). Role of exopolysaccharide in salt stress resistance and cell motility of mesorhizobium alhagi CCNWXJ12–2T. Appl. Microbiol. Biotechnol. 101, 2967–2978. doi: 10.1007/s00253-017-8114-y
Liu X., Zhu H., Song H., Wang Q., Xiong X., Wu C., et al. (2018b). Euchlorocystis gen. nov. and Densicystis gen. nov., two new genera of oocystaceae algae from high-altitude semi-saline habitat (Trebouxiophyceae, Chlorophyta). J. Eukaryot. Microbiol. 65, 200–210. doi: 10.1111/jeu.12455
Pancha I., Chokshi K., Maurya R., Trivedi K., Patidar S. K., Ghosh A., et al. (2015). Salinity induced oxidative stress enhanced biofuel production potential of microalgae scenedesmus sp. CCNM 1077. Bioresource Technology. 189, 341–348. doi: 10.1016/j.biortech.2015.04.017
Park S., Kim M., Lee S. G., Lee L., Choi H. K., Jin E. (2015). Contrasting photoadaptive strategies of two morphologically distinct dunaliella species under various salinities. J. Appl. Phycol. 27, 1053–1062. doi: 10.1007/s10811-014-0394-3
Qurashi A. W., Sabri A. N. (2012). Bacterial exopolysaccharide and biofilm formation stimulate chickpea growth and soil aggregation under salt stress. Braz. J. Microbiol. 43, 1183–1191. doi: 10.1590/S1517-83822012000300046
Rasool S., Hameed A., Azooz M., Rehman M., Siddiqi T., Ahmad P., et al. (2013). “Salt stress: causes, types and responses of plants,” in Ecophysiology and responses of plants under salt stress. Eds. Ahmad P., Azooz M., Prasad M. (Springer, New York, NY). doi: 10.1007/978–1-4614–4747-4_1
Ren J. (2021). Growth, biochemical components, and transcriptome analysis of oocystis borgei preserved under different light levels. [Master dissertation]. Guangdong Ocean University, zhanjiang.
Ren J. J., Hong T., Zhang N., Li C. L. (2020). Effect of salinity on chlorophyll fluorescence parameters of oocystis borgei and cloning and expression analysis of psba gene. J. Guangdong Ocean University. 40, 30–39. doi: 10.3969/j.issn.1673–9159.2020.03.005
Rinaudi L. V., González J. E. (2009). The low-molecular-weight fraction of exopolysaccharide II from sinorhizobium meliloti is a crucial determinant of biofilm formation. J. Bacteriol 191, 7216–7224. doi: 10.1128/JB.01063-09
Salam K. A., Velasquez-Orta S. B., Harvey A. P. (2016). A sustainable integrated in situ transesterification of microalgae for biodiesel production and associated co-product-A review. Renew. Sustain. Energy Rev. 65, 1179–1198. doi: 10.1016/j.rser.2016.07.068
Sedjati S., Santosa G. W., Yudiati E., Supriyantini E., Ridlo A., Kimberly F. D. (2019). “Chlorophyll and carotenoid content of dunaliella salina at various salinity stress and harvesting time,” in IOP Conference Series: Earth and Environmental Science, Vol. 246. 012025. 4th International Conference on Tropical and Coastal Region Eco Development, 30–31 October 2018. Semarang, Indonesia. IOP Publishing Ltd, Philadelphia, PA. doi: 10.1088/1755–1315/246/1/012025
Smyth K., Elliott M. (2016). “Effects of changing salinity on the ecology of the marine environment,” in Stressors in the marine environment: physiological and ecological responses; societal implication. Eds. Martin S., Nia W. (Oxford Academic, New York, NY, Oxford). doi: 10.1093/acprof:oso/9780198718826.003.0009
Srivastava G., Goud V. V. (2017). Salinity induced lipid production in microalgae and cluster analysis (ICCB 16-BR_047). Bioresource Technol. 242, 244–252. doi: 10.1016/j.biortech.2017.03.175
Takeuchi T., Utsunomiya K., Kobayashi K., Owada M., Karube I. (1992). Carbon dioxide fixation by A unicellular green alga Oocystis sp. J. Biotechnol. 25, 261–267. doi: 10.1016/0168–1656(92)90160-B
Talebi A. F., Meisam T., Seyed K. M., Masoud T., Foad M. (2013). Comparative salt stress study on intracellular ion concentration in marine and salt-adapted freshwater strains of microalgae. Notulae Botanicae Horti Agrobotanici Cluj-napoca 5, 309–315. doi: 10.15835/nsb539114
Talukdar J., Kalita M. C., Goswami B. C. (2012). Effects of salinity on growth and total lipid content of the biofuel potential microalga ankistrodesmus falcatus (Corda) ralfs. Int. J. Sci. Eng. Res. 3, 1–7.
Tewari S., Arora N. K. (2014). Multifunctional exopolysaccharides from pseudomonas aeruginosa PF23 involved in plant growth stimulation, biocontrol and stress amelioration in sunflower under saline conditions. Curr. Microbiol. 69, 484–494. doi: 10.1007/s00284-014-0612-x
Wang H., Fu R., Huang B. (2012). Rapid determination of lipid in chlorella based on nile red fluorescence. China Oils Fats. 37, 78–81. doi: 10.3969/j.issn.1003–7969.2012.03.020
Wang X. Q., Zhang Y. L., Li C. L., Huang X. H., Li F., Wang X. Y., et al. (2022). Allelopathic effect of oocystis borgei culture on microcystis aeruginosa. Environ. Technol. 43 (11), 1662–1671. doi: 10.1080/09593330.2020.1847202
Xie L., Lin X., Xu Z., Hu R., Qi X., Cheng K. (2003). Phytoplankton communities and physical and chemical factors in different types of shrimp ponds. Ecologic Sci. 22, 034–037. doi: 10.3969/j.issn.1008–8873.2003.01.009
Xie L. S., Zhang N., Li C. L., Huang X. H. (2020). Effects of sodium nitrate on growth, biochemical components, and sedimentation of oocystis borgei. J. Guangdong Ocean University. 40, 48–55. doi: 10.3969/j.issn.1673–9159.2020.03.007
Yang A., Zhang G., Meng F., Zhi R., Zhang P., Zhu Y. (2019). Nitrogen metabolism in photosynthetic bacteria wastewater treatment: A novel nitrogen transformation pathway. Bioresour. Technol. 294, 122162. doi: 10.1016/j.biortech.2019.122162
Yao C. H., Ai J. N., Cao X. P., Xue S. (2013). Salinity manipulation as an effective method for enhanced starch production in the marine microalga tetraselmis subcordiformis. Bioresource Technology. 146, 663–671. doi: 10.1016/j.biortech.2013.07.134
Zhang T., Gong H. M., Wen X. G., Lu C. M. (2010). Salt stress induces a decrease in excitation energy transfer from phycobilisomes to photosystem ii but an increase to photosystem I in the cyanobacterium Spirulina platensis. J. Plant Physiol. 167, 951–958. doi: 10.1016/j.jplph.2009.12.020
Zhang L., Pei H., Chen S., Jiang L., Hou Q., Yang Z. (2018). Salinity-induced cellular cross-talk in carbon partitioning reveals starch-to-lipid biosynthesis switching in low-starch freshwater algae. Bioresour Technol. 250, 449–456. doi: 10.1016/j.biortech.2017.11.067
Keywords: Euchlorocystis marina, salinity, adaptation, growth, physiology, biochemical composition
Citation: Pan Y, Amenorfenyo DK, Dong M, Zhang N, Huang X, Li C and Li F (2024) Effects of salinity on the growth, physiological and biochemical components of microalga Euchlorocystis marina. Front. Mar. Sci. 11:1402071. doi: 10.3389/fmars.2024.1402071
Received: 16 March 2024; Accepted: 26 April 2024;
Published: 10 May 2024.
Edited by:
Yenny Risjani, University of Brawijaya, IndonesiaReviewed by:
Mai-Lan Pham, University for Continuing Education Krems, AustriaHongli Cui, Chinese Academy of Sciences (CAS), China
Copyright © 2024 Pan, Amenorfenyo, Dong, Zhang, Huang, Li and Li. This is an open-access article distributed under the terms of the Creative Commons Attribution License (CC BY). The use, distribution or reproduction in other forums is permitted, provided the original author(s) and the copyright owner(s) are credited and that the original publication in this journal is cited, in accordance with accepted academic practice. No use, distribution or reproduction is permitted which does not comply with these terms.
*Correspondence: Feng Li, lifeng2318@gdou.edu.cn
†These authors have contributed equally to this work and share first authorship